10 Optimizing Home Compostable Polymer Blends: Compatibility and Performance Parameters
Optimizing Home Compostable Polymer Blends: Compatibility and Performance Parameters
Mauricio Ruiz
1. Introduction to biodegradable plastics
The growing environmental concerns surrounding plastic waste have led to increased interest in biodegradable and compostable polymers as sustainable alternatives to conventional plastics. Among these, home compostable polymers offer a promising solution by enabling consumers to responsibly dispose of plastic products in their own backyard compost bins. However, developing high-performance home compostable materials that meet both technical and environmental requirements remains a significant challenge.
1.1 Polymer Blends
This book chapter explores recent advancements on the critical factors involved in optimizing polymer blends for home compostability while maintaining desirable material properties and performance. By blending different biodegradable polymers, it is possible to tailor the characteristics of the resulting material. Achieving compatibility between blend components and balancing properties like mechanical strength, barrier performance, and degradation rate requires careful consideration of multiple parameters such as:
- Polymer selection and compatibility assessment
- Processing techniques for optimal blend morphology
- Characterization methods for evaluating blend properties
- Degradation mechanisms and kinetics in home composting conditions
- Performance requirements for various applications
- Regulatory standards and certification processes
- Life cycle assessment and sustainability considerations
By providing a comprehensive overview of the scientific principles, technical approaches, and practical considerations involved, this book aims to guide researchers, engineers, and product developers in creating innovative home compostable materials.
1.2 Video: Our Plastic Predicament – To Bio… or Not to Bio?
2. Key Concepts in Home Compostable Polymer Blends
2.1 Biodegradation vs. Compostability
Biodegradation refers to the breakdown of materials by microorganisms like bacteria and fungi into water, carbon dioxide, and biomass. Compostability is a more specific form of biodegradation that occurs under composting conditions and results in the formation of compost.
For a material to be considered home compostable, it must biodegrade in a home composting environment within a reasonable timeframe (typically 6-12 months) without leaving behind toxic residues or microplastics. This is in contrast to industrial composting, which occurs under more controlled conditions with higher temperatures.
2.2 Polymer Blending
Polymer blending involves mixing two or more polymers to create a new material with combined properties. For biodegradable polymers, blending is often used to:
– Improve mechanical properties
– Enhance processability
– Accelerate biodegradation rates
– Reduce costs
Common biodegradable polymers used in blends include:
– Polylactic acid (PLA)
– Poly-L-lactic acid (PLLA)
– Poly(glycolic acid) (PGA)
– Polybutylene succinate (PBS)
– Polycaprolactone (PCL)
– Polyhydroxyalkanoates (PHAs)
– Polybutylene adipate terephthalate (PBAT)
– Thermoplastic starch (TPS)
Polymer blending can effectively tailor the disintegration rate of biodegradable polymers in soil.
- Poly(hydroxybutyrate-co-hydroxyvalerate) (PHBV) biodegradation can be accelerated by blending with poly(butylene succinate-co-adipate) (PBSA) and polycaprolactone (PCL).
- Poly(butylene succinate) (PBS) showed high disintegration rates in soil, contrary to its market reputation as non-biodegradable in soil.
- PBS biodegradation rate is highly dependent on specific soil conditions.
- PBS biodegradation can be substantially accelerated by blending with PBSA.
- Poly(lactic acid) (PLA) disintegration and overall biodegradation in soil can be enhanced by blending with PCL.
- PLA/PCL blends showed 39% conversion to CO2 in 12 months in soil and 89% conversion in 6 months under home composting conditions.
2.3 Compatibility and Phase Morphology
The compatibility between polymers in a blend significantly impacts its properties and biodegradation behavior. Incompatible blends tend to have a heterogeneous phase morphology with distinct domains of each polymer. This can lead to poor mechanical properties but may accelerate biodegradation by creating more interfaces for microbial attack.
Compatible blends have a more homogeneous morphology. While this typically improves mechanical properties, it can slow biodegradation if the more resistant polymer encapsulates the more readily degradable one.
2.4 Crystallinity
The degree of crystallinity in polymers and polymer blends affects both their mechanical properties and biodegradation rates. Highly crystalline regions are more resistant to microbial attack compared to amorphous regions. Blending can alter the crystallization behavior of polymers, potentially impacting biodegradation.
2.5 Interfacial Adhesion and Compatibilizers
In incompatible blends, poor interfacial adhesion between polymer phases can lead to weak mechanical properties. Compatibilizers are additives used to improve interfacial adhesion and overall blend properties. However, their impact on biodegradation must be carefully considered.
Mixing polymers with inorganic fillers like hydroxyapatite (HAP), talc, or calcium carbonate (CaCO3) can enhance biodegradability or home compostability by creating pores and increasing surface area as the filler degrades, allowing for faster breakdown of the polymer matrix.
Mixing with other biodegradable polymers enhances the degradability. An example may be the blend of poly(glycolic acid) (PGA) with polylactic acid (PLA), enhancing the degradability of the PLA/PHA (polyhydroxyalkanoates) mixture. This is due to several key factors:
- Faster Hydrolysis Rate: PGA has a higher rate of hydrolysis compared to PLA. When blended, PGA degrades more quickly, releasing glycolic acid, which can act as a catalyst for the hydrolysis of PLA. This catalytic effect accelerates the breakdown of PLA chains, leading to faster overall degradation of the blend.
- Acidic Environment: The degradation of PGA produces acidic byproducts that lower the pH of the surrounding environment. This acidic condition further promotes the hydrolysis of ester bonds in PLA, enhancing its degradation rate.
- Increased Surface Area: As PGA degrades, it creates pores and increases the surface area of the remaining polymer matrix. This structural change allows for greater microbial access and interaction with the polymer, facilitating faster biodegradation.
- Synergistic Effects: The combination of PGA and PLA can lead to synergistic effects where the presence of one polymer enhances the degradation characteristics of the other, making it more effective than either polymer alone.
2.6 Other Environmentally Friendly Substances
In addition to PGA, there are several other environmentally friendly substances that can provide similar effects in enhancing biodegradability:
- Polycaprolactone (PCL): Blending PCL with PLA can improve biodegradability due to PCL’s faster degradation rate and its ability to create a more hydrophilic environment.
- Starch: Incorporating thermoplastic starch can enhance microbial activity and increase the overall biodegradation rate due to its natural biodegradability and ability to attract microorganisms.
- Chitosan: This biopolymer derived from chitin has antimicrobial properties and can accelerate degradation when blended with other biodegradable polymers.
- Cellulose Nanocrystals: These can reinforce biodegradable polymers while also promoting degradation through increased surface area and hydrophilicity.
- Natural Fibers: Adding natural fibers (like jute or hemp) not only improves mechanical properties but also enhances biodegradability by providing additional surfaces for microbial colonization.
These substances can be effectively used in blends or composites with biodegradable polymers to create materials that are not only functional but also environmentally friendly, contributing to reduced plastic waste and improved sustainability.
2.6 Video: Blending and Degradation Analysisi of Heat Sensitive Biodegradable Polymer.
3. Biodegradation Key Theories and Principles
Home compostable polymer blends offer a promising approach to reducing plastic waste and closing material loops. However, their development requires a deep understanding of polymer science, microbiology, and materials engineering. By carefully considering the concepts, theories, and principles of biodegradation, researchers and engineers can create innovative blends that meet both performance and environmental requirements.
3.1 Biodegradation Mechanisms.
The biodegradation of polymer blends typically occurs through a two-step process:
a) Depolymerization: Extracellular enzymes from microorganisms break down the polymer chains into smaller fragments (oligomers and monomers).
b) Mineralization: The small fragments are taken up by microorganisms and converted into biomass, CO2, and water (in aerobic conditions) or methane (in anaerobic conditions).
The rate-limiting step is often the initial depolymerization, particularly for synthetic polymers that microorganisms have not evolved to degrade efficiently.
3.2 Factors Affecting Biodegradation Rates
Several factors influence the biodegradation rate of polymer blends:
– Chemical structure and composition
– Molecular weight
– Crystallinity
– Surface area and particle size
– Environmental conditions (temperature, moisture, pH, microbial population)
– Presence of additives or impurities
3.3 Synergistic and Antagonistic Effects in Blends.
The biodegradation of polymer blends is not always a simple sum of the degradation rates of individual components. Synergistic effects can occur when:
– One polymer creates pathways for microbial attack on the other.
– Degradation products of one polymer catalyze the breakdown of the other.
– Blending alters the morphology in a way that enhances overall degradation.
Antagonistic effects may arise if:
– A more resistant polymer encapsulates or protects a more degradable one.
– Degradation products inhibit microbial activity.
– Blending increases overall crystallinity.
3.5 Role of Interfaces in Biodegradation
Interfaces between different polymer phases in a blend can play a crucial role in biodegradation:
– They may act as preferential sites for microbial attachment and enzyme action.
– Incompatible blends with more interfaces may degrade faster initially.
– However, very fine dispersion of phases can sometimes hinder biodegradation.
3.6 Importance of Standardized Testing
Standardized testing methods are essential for evaluating and comparing the industrial and home compostability of polymer blends. Key standards include:
– EN13432: European Harmonized standard for compostable packaging. The testing is performed under controlled composting conditions for industrial composting, where temperature and moisture are controlled.
– AS 5810 (Australia): “Biodegradable plastics suitable for home composting”
– NF T51-800 (France): “Plastics — Specifications for plastics suitable for home composting”
These standards typically assess:
– Biodegradation rate (e.g., 90% in 12 months at 20-30°C)
– Disintegration (physical breakdown)
– Ecotoxicity of residues and resulting compost
– Heavy metal content and other potential contaminants
3.7 Balancing Performance and Biodegradability
A key challenge in developing home compostable polymer blends is balancing functional performance with biodegradability. This often involves trade-offs, as properties that enhance material durability during use may hinder biodegradation at end-of-life.
Strategies to address this challenge include:
– Using compatibilizers that enhance properties but do not inhibit biodegradation
– Developing multi-phase morphologies that maintain performance while allowing microbial access
– Incorporating bio-based additives that enhance both properties and biodegradation
3.8 Life Cycle Considerations
When developing home compostable polymer blends, it’s important to consider the entire life cycle of the material:
– Raw material sourcing (renewable vs. fossil-based)
– Energy and resources used in production
– Performance during use phase
– End-of-life management options
– Overall environmental impact compared to alternatives
Life Cycle Assessment (LCA) tools can help evaluate the sustainability of different blend formulations and guide development efforts.
4. Paper review: “Acceleration of Biodegradation Using Polymer Blends and Composites”
The article titled “Acceleration of Biodegradation Using Polymer Blends and Composites” by Easton et al. examines strategies to enhance the biodegradation rates of bioplastics through blending with other polymers or incorporating fillers to create composites.
The authors discuss various applications of biodegradable polymer blends and composites, including tissue engineering, packaging, and agriculture. They focus on methods to control and accelerate biodegradation, such as promoting local acid hydrolysis, attracting microbes, and creating pores or phase separation. These connections demonstrate how fundamental principles of polymer solution and solid behavior underpin the design and performance of biodegradable polymer blends and composites.
4.1 Key concepts of Polymer solution and solid behavior:
4.1.1 Polymer blends: The article extensively discusses polymer blends, which directly connects to principles of polymer miscibility and phase behavior. For example, the authors note that polymer blends can be miscible (homogeneous) or immiscible (heterogeneous) depending on the compatibility of the constituent polymers. This aligns with fundamental concepts of polymer thermodynamics and phase diagrams.
4.1.2 Crystallinity: The paper mentions how the crystallinity of polymers like PLA affects their degradation rates. This relates to principles of polymer crystallization and how it influences material properties.
4.1.3 Glass Transition Temperature: The authors discuss how blending affects the glass transition temperature (Tg) of polymer systems. This connects to the concept of Tg in polymer physics and how it relates to polymer chain mobility and material properties.
4.1.4 Interfacial Adhesion: The paper discusses the use of compatibilizers to improve interfacial adhesion between immiscible polymers. This relates to principles of polymer interfaces and surface tension.
4.1.5 Diffusion and Permeability: The authors mention how the addition of fillers or creation of pores can affect water absorption and diffusion into the polymer matrix, which directly relates to principles of mass transport in polymers.
4.1.6 Polymer Degradation Mechanisms: The paper extensively covers hydrolysis and enzymatic degradation of polymers, which connects to principles of polymer chemical stability and reaction kinetics.
4.1.7 Composite Materials: The discussion of polymer composites relates to principles of filler dispersion, interfacial interactions, and how they affect bulk material properties.
4.2 Relevant article figures
I selected figures 1, 2, 3 and 5 as the most relevant ones for understanding the acceleration of biodegradation using polymer blends and composites. These figures collectively provide a comprehensive view of the structural basis, mechanisms, and experimental evidence for accelerated biodegradation in polymer blends and composites. Here is an explanation of each one:
4.2.1 Figure 1 of the article
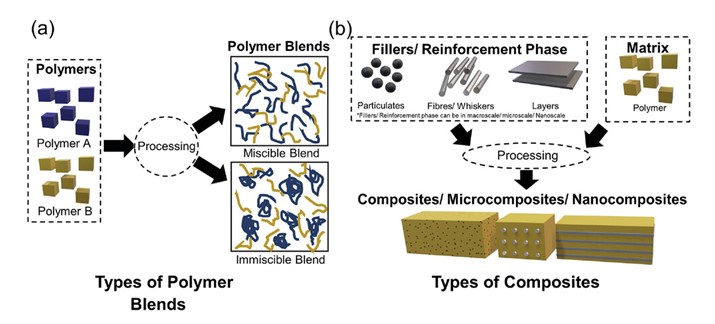
The figure shows the structural organization of blends versus composites. This visual representation helps to explain how these materials are constructed and how their properties might differ as a result of their distinct structures. The main topics to highlight are:
4.2.1.1 Polymer Blends
The left side of Figure 1 (part a) depicts polymer blends:
- Miscible blends: These show a homogeneous mixture of two polymers, resulting in a single-phase system with uniform properties throughout.
- Immiscible blends: These display a two-phase system where the polymers remain separate due to high interfacial tension. This can lead to distinct domains of each polymer within the blend.
4.2.1.2 Polymer Composites
The right side of Figure 1 (part b) show polymer composites:
- Microcomposites: These contain larger filler particles or fibers dispersed within the polymer matrix.
- Nanocomposites: These feature nanoscale fillers (such as nanoparticles, nanofibers, or nanoplatelets) distributed throughout the polymer matrix.
4.2.3 Figure 2 of the article.
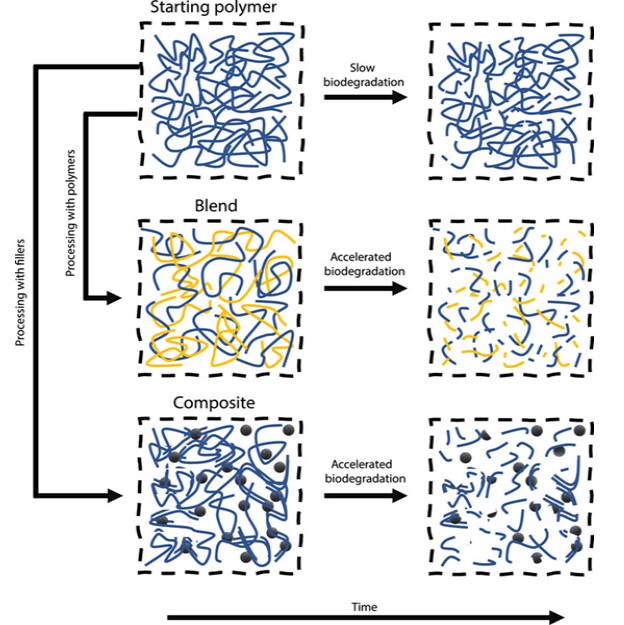
Figure 2 of the selected article illustrates the process of accelerated biodegradation in polymer blends and composites. This visual representation demonstrates how the addition of a faster-degrading component (either as a blend or filler) can lead to accelerated biodegradation of the entire material system. The key mechanism illustrated is the creation of increased surface area through the initial degradation of one component, which then facilitates faster breakdown of the remaining polymer matrix.
This figure schematically shows how blending and filler addition can accelerate biodegradation. It illustrates:
- The initial state of the polymer
- The degradation of the filler or blend component
- The resulting increased surface area
- The accelerated degradation of the remaining polymer
4.2.4 Figure 3 of the article.
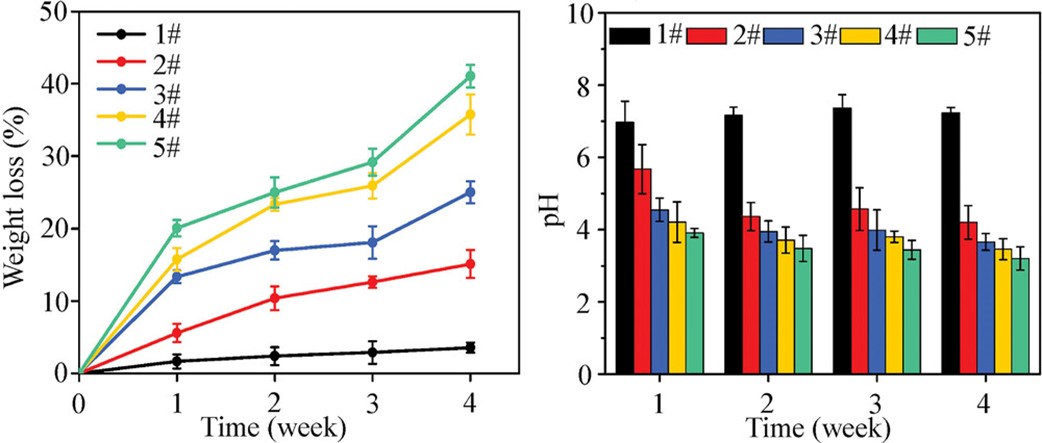
Figure 3 in the article illustrates the degradation behavior of PLLA/HAP/PGA blends over a 28-day period in a phosphate buffered saline (PBS) solution at pH 7.4 and 37°C.
The left graph demonstrates that as the PGA content increases, the weight loss percentage also increases. The blend with the highest PGA content (0/10/90) shows the most rapid and significant weight loss, reaching about 25% after 28 days. In contrast, the blend without PGA (90/10/0) shows minimal weight loss of only about 3.3% after 28 days.
The graph in the right displays the changes in pH of the surrounding PBS solution during the degradation process. The same color coding is used for the different blend compositions.The pH graph reveals that as PGA content increases, the pH of the solution decreases more rapidly and to a lower value. This is due to the faster degradation of PGA, which releases acidic byproducts. The blend with the highest PGA content (0/10/90) causes the pH to drop to about 6.5 after 28 days, while the blend without PGA (90/10/0) maintains a relatively stable pH close to the initial value.
In summary, these results demonstrate that blending PGA into the PLLA/HAP scaffold accelerates the degradation process. The increased weight loss and decreased pH are attributed to the faster hydrolysis of PGA compared to PLLA, and the subsequent creation of an acidic environment that further catalyzes the degradation of the entire scaffold.
The last figure of the article that I chose to explain is figure no. 5.
4.2.4 Figure 5 of the article.
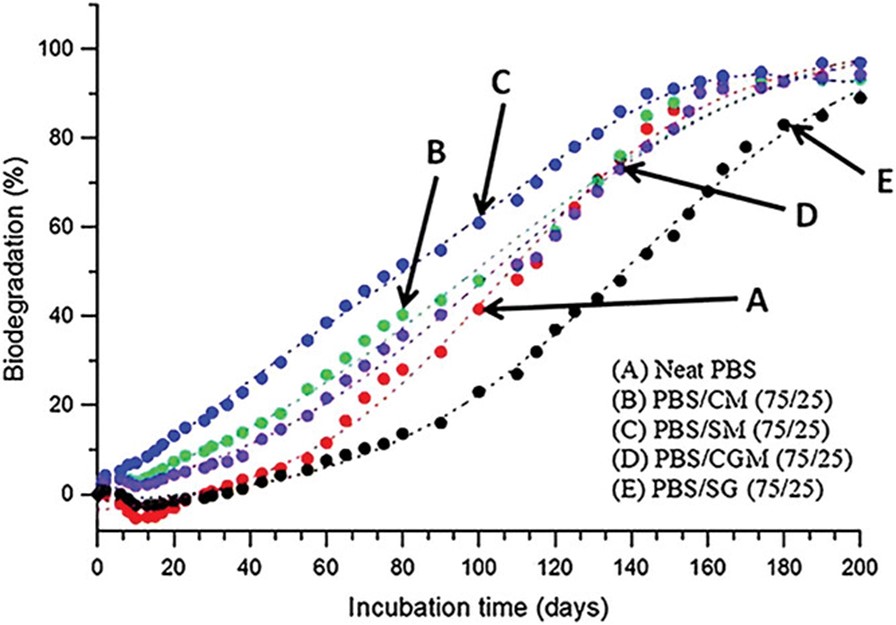
Figure 5 illustrates the biodegradation behavior of polybutylene succinate (PBS) and PBS bio-composites according to the ASTM D6400 standard.The graph shows biodegradation percentage over incubation time for different materials:
A. Pure PBS
B. PBS/CM (Canola Meal) composite
C. PBS/SM (Soy Meal) composite
D. PBS/CGM (Corn Gluten Meal) composite
E. PBS/SG (Switchgrass) compositeKey observations from the graph:
- All bio-composites (B-E) show faster initial biodegradation rates compared to pure PBS (A).
- The PBS/SM composite (C) exhibits the fastest overall biodegradation, reaching 90% biodegradability in approximately 140 days.
- The PBS/CM (B) and PBS/CGM (D) composites show similar biodegradation profiles, achieving 90% biodegradability in about 160 days.
- The PBS/SG composite (E) initially degrades faster than pure PBS but then slows down, taking about 200 days to reach 90% biodegradability.
- Pure PBS (A) has the slowest biodegradation rate, not reaching 90% biodegradability within the timeframe shown.
The accelerated biodegradation of the composites (except for PBS/SG) is attributed to the presence of protein-rich meals in the bio-fillers, which enhance initial microorganism growth in the compost. This leads to faster hydrolysis of the PBS polymer matrix.The slower degradation of the PBS/SG composite, despite its initial rapid degradation, is due to its high lignin content. Lignin requires oxidative degradation rather than enzymatic hydrolysis, which slows down the overall biodegradation process.This figure demonstrates how blending PBS with different bio-fillers can significantly influence its biodegradation rate, allowing for tailored degradation profiles for various applications.
5. Main Takeaways
- Blending biodegradable polymers or adding biodegradable fillers can effectively speed up the overall degradation rate of bioplastics.
- Various mechanisms can be used to accelerate biodegradation:
- Promoting local acid hydrolysis
- Attracting microbes for faster breakdown
- Creating pores to increase surface area
- Inducing phase separation
- The choice of blend components or fillers can be tailored to specific applications and desired degradation rates.
- Accelerating biodegradation can help prevent microplastic formation and reduce environmental impact.
5.1 Real-World Implications
Think of these biodegradable blends and composites like a compost pile in your backyard. Just as adding the right mix of “green” and “brown” materials can speed up composting, combining different biodegradable polymers or adding specific fillers can accelerate the breakdown of bioplastics. For example:
- A biodegradable shopping bag made from a polymer blend might break down in months rather than years, reducing litter and environmental harm.
- A medical implant composed of a carefully designed composite could provide support for healing, then safely dissolve in the body once its job is done.
- Agricultural films made from these materials could protect crops during the growing season, then decompose into the soil without leaving harmful residues.
By fine-tuning the ingredients in these “polymer recipes,” scientists can create materials that perform their intended function, then break down quickly and safely when no longer needed. This approach brings us closer to a world where plastic products don’t outlive their usefulness by hundreds of years.
6.0 INTERACTIVE ELEMENTS
6.1 VIDEO: The Game Show – Our plastic predicament.
6.2 Test Your Knowledge: Biodegradable Polymer Blends and Composites
7.0 Supplemental information
7.1 Biopolymers infographic
7.2 Presentation on Sustainable Polymers
8.0 Bibliography
- Link to video: To Bio or Not To Bio | Our Plastic Predicament: Episode 5 #ThinkBioplastic https://www.youtube.com/watch?v=utuEaMN8im8&t=282s ↵
- Bibliography: Kale G, Kijchavengkul T, Auras R, Rubino M, Selke SE, Singh SP. Compostability of bioplastic packaging materials: an overview. Macromolecular Bioscience. 2007;7(3):255-277. ↵
- Muthuraj R, Misra M, Mohanty AK. Biodegradable compatibilized polymer blends for packaging applications: A literature review. Journal of Applied Polymer Science. 2018;135(24):45726. ↵
- Narancic T, Verstichel S, Reddy Chaganti S, et al. Biodegradable plastic blends create new possibilities for end-of-life management of plastics but they are not a panacea for plastic pollution. Environmental Science & Technology. 2018;52(18):10441-10452. ↵
- Luzi F, Torre L, Kenny JM, Puglia D. Bio-based and biodegradable polymers for food packaging: A review. Frontiers in Materials. 2019;6:206. ↵
- Ferreira FV, Cividanes LS, Gouveia RF, Lona LM. An overview on properties and applications of poly(butylene adipate-co-terephthalate)–PBAT based composites. Polymer Engineering & Science. 2019;59(s2):E7-E15. ↵
- Link to video: Blending and Degradation Analysis of the Heat Sensitive Biodegradable Polymer https://www.youtube.com/watch?v=roAl0VdqAM0&t=194s ↵
- Bibliography: Emadian, S. M., Onay, T. T., & Demirel, B. (2017). Biodegradation of bioplastics in natural environments. Waste Management, 59, 526-536. ↵
- Kale, G., Kijchavengkul, T., Auras, R., Rubino, M., Selke, S. E., & Singh, S. P. (2007). Compostability of bioplastic packaging materials: an overview. Macromolecular Bioscience, 7(3), 255-277. ↵
- Muthuraj, R., Misra, M., & Mohanty, A. K. (2018). Biodegradable compatibilized polymer blends for packaging applications: A literature review. Journal of Applied Polymer Science, 135(24), 45726. ↵
- The publicly available abstract for this paper can be found at: https://onlinelibrary.wiley.com/doi/10.1002/macp.202200421 ↵
- Link to video The Game Show - Our plastic predicament: https://www.youtube.com/watch?v=5r8EvaXI9yU ↵