17 Chapter 17 – Alcohols and Phenols Solutions to Problems
17.4 |
|
 |
|
(a) |
HC≡CH < |
(CH3)2CHOH< |
CH3OH < |
(CF3)2CHOH |
|
|
alkyne |
hindered alcohol |
alcohol |
alcohol with electron-withdrawing groups |
|
|
|
|
|
|
|
(b) |
p-Methylphenol < |
Phenol < |
p-(Trifluoromethyl)phenol |
|
|
phenol with electron-donating groups |
|
phenol with electron-withdrawing groups |
|
|
|
|
|
|
(c) |
Benzyl alcohol < |
Phenol < |
p-Hydroxybenzoic acid |
|
|
alcohol |
|
carboxylic acid |
17.5 |
We saw in Chapter 16 that a nitro group is electron-withdrawing. Since electron-withdrawing groups stabilize anions, p-nitrobenzyl alcohol is more acidic than benzyl alcohol. The methoxyl group, which is electron-donating, destabilizes an alkoxide ion, making p-methoxybenzyl alcohol less acidic than benzyl alcohol. |
17.6 |
(a) |
In a hydroboration/oxidation reaction, the hydroxyl group is bonded to the less substituted carbon.
|
|
(b) |
Markovnikov product results from oxymercuration/reduction
|
|
(c) |
Hydroxylation results in a diol with syn stereochemistry.
|
17.7 |
(a) |
NaBH4 reduces aldehydes and ketones without interfering with other functional groups.
|
|
(b) |
LiAlH4, a stronger reducing agent, reduces both ketones and esters.
|
|
(c) |
LiAlH4 reduces carbonyl functional groups without reducing double bonds.
|
17.9 |
All of the products have an –OH and a methyl group bonded to what was formerly a ketone carbon. |
|
(a) |
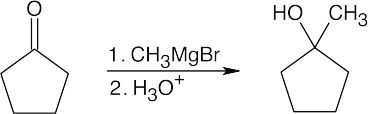 |
|
(b) |
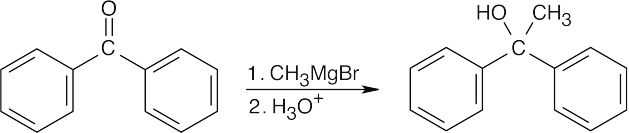 |
|
(c) |
 |
17.10 |
First, identify the type of alcohol. If the alcohol is primary, it can only be synthesized from formaldehyde plus the appropriate Grignard reagent. If the alcohol is secondary, it is synthesized from an aldehyde and a Grignard reagent. (Usually, there are two combinations of aldehyde and a Grignard reagent). A tertiary alcohol is synthesized from a ketone and a Grignard reagent. If all three groups on the tertiary alcohol are different, there are often three different combinations of ketone and a Grignard reagent. If two of the groups on the alcohol carbon are the same, the alcohol may also be synthesized from an ester and two equivalents of Grignard reagents. |
|
(a) |
2-Methyl-2-propanol is a tertiary alcohol. To synthesize a tertiary alcohol, start with a ketone.
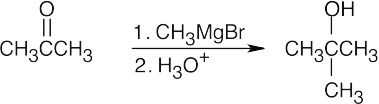
If two or more alkyl groups bonded to the carbon bearing the –OH group are the same, an alcohol can be synthesized from an ester and a Grignard reagent.
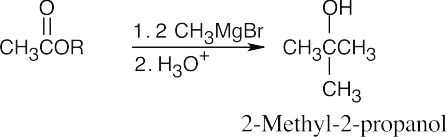
|
|
(b) |
Since 1-methylcyclohexanol is a tertiary alcohol, start with a ketone.
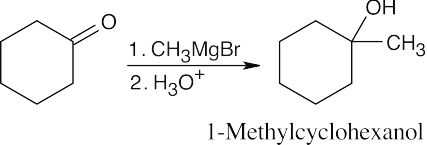
|
|
(c) |
3-Methyl-3-pentanol is a tertiary alcohol. When two of the three groups bonded to the alcohol carbon are the same, either a ketone or an ester can be used as a starting material.
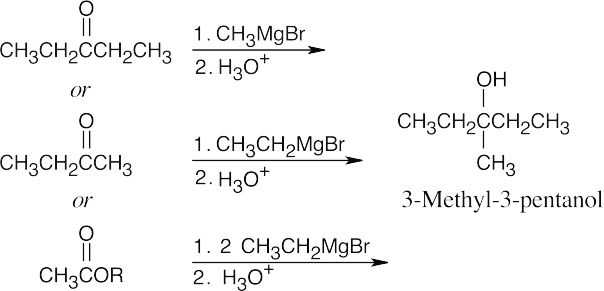
|
|
(d) |
Three possible combinations of ketone plus a Grignard reagent can be used to synthesize this tertiary alcohol.
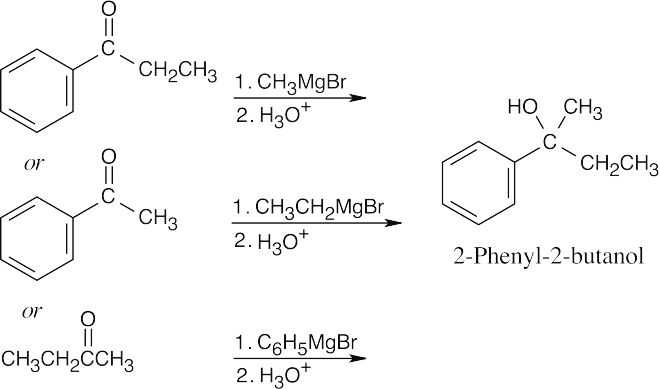
|
|
(e) |
Formaldehyde must be used to synthesize this primary alcohol.
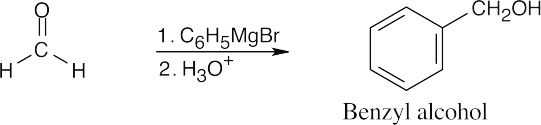
|
|
(f) |
As in (e), use formaldehyde to synthesize a primary alcohol.

|
17.11 |
First, interpret the structure of the alcohol. This alcohol, 1-ethylcyclohexanol, is a tertiary alcohol that can be synthesized from a ketone. Only one combination of ketone and a Grignard reagent is possible.

|
17.12 |
Recall from Chapter 11 that –OH is a very poor leaving group in reactions run under SN2 conditions. A toluenesulfonate, however, is a very good leaving group, and reaction of the toluenesulfonate of the alcohol with –CN proceeds readily under SN2 conditions to give the desired product with inversion of configuration at the chirality center.
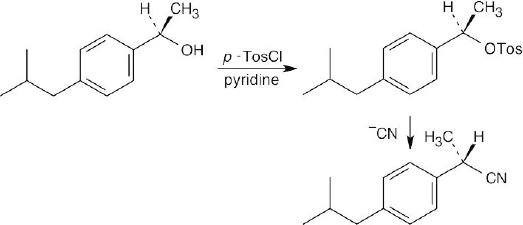
|
17.14 |
Aldehydes are synthesized from oxidation of primary alcohols, and ketones are synthesized from oxidation of secondary alcohols. |
|
(a) |
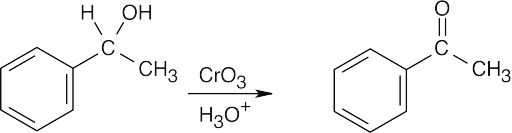 |
|
(b) |
 |
|
(c) |
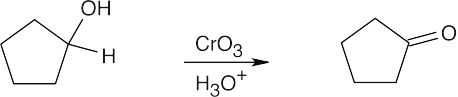 |
17.15 |
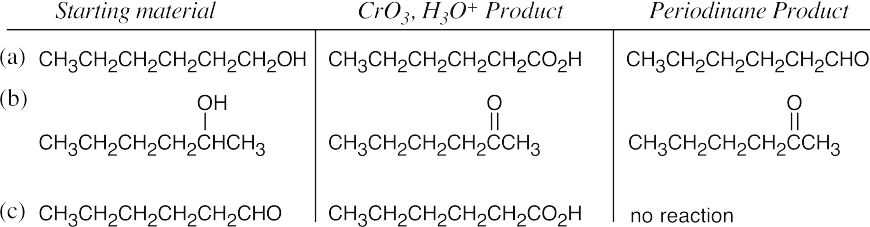 |
17.16 |
This is an SN2 reaction in which the nucleophile F– attacks silicon and displaces an alkoxide ion as the leaving group. |
17.17 |
Phosphoric acid protonates 2-methylpropene, forming a tert-butyl carbocation.
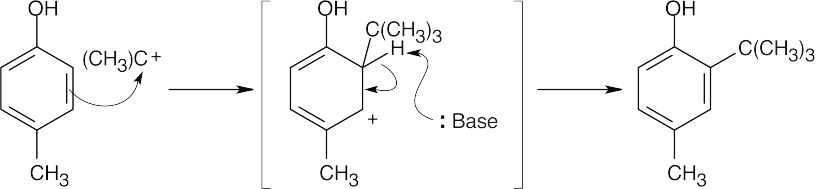
The tert-butyl carbocation acts as an electrophile and alkylates p-cresol. Alkylation occurs ortho to the –OH group for both steric and electronic reasons.
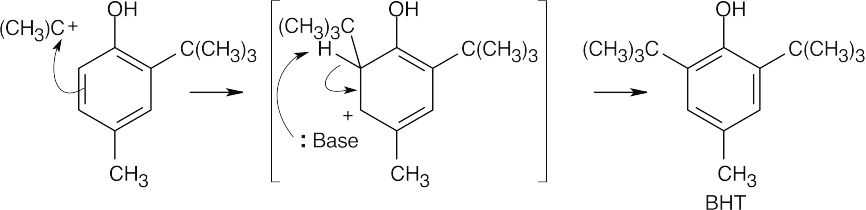
A second tert-butyl carbocation alkylation forms BHT.
|
17.18 |
The infrared spectra of cholesterol and 5-cholestene-3-one each exhibit a unique absorption that makes it easy to distinguish between them. Cholesterol shows an –OH stretch at 3300- 3600 cm–1, and 5-cholestene-3-one shows a C=O stretch at 1715 cm–1. In the oxidation of cholesterol to 5-cholestene-3-one, the –OH band disappears and is replaced by a C=O band. When oxidation is complete, no –OH absorption should be visible. |
17.19 |
Under conditions of slow exchange, the –OH signal of a tertiary alcohol (R3COH) is unsplit, the signal of a secondary alcohol (R2CHOH) is split into a doublet, and the signal of a primary alcohol (RCH2OH) is split into a triplet. |
|
(a) |
2-Methyl-2-propanol is a tertiary alcohol; its –OH signal is unsplit. |
|
|
(b) |
Cyclohexanol is a secondary alcohol; its –OH absorption is a doublet. |
|
|
(c) |
Ethanol is a primary alcohol; its –OH signal appears as a triplet. |
|
|
(d) |
2-Propanol is a secondary alcohol; its –OH absorption is split into a doublet. |
|
|
(e) |
Cholesterol is a secondary alcohol; its –OH absorption is split into a doublet. |
|
|
(f) |
1-Methylcyclohexanol is a tertiary alcohol; its –OH signal is unsplit. |
|
Additional Problems
Visualizing Chemistry
17.27 |
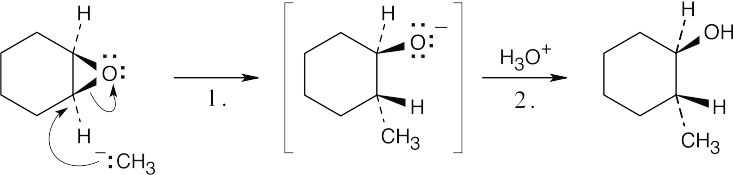 |
|
Step 1: |
SN2 reaction of Grignard reagent. |
|
Step 2: |
Protonation of alkoxide oxygen. |
|
The methyl group and the hydroxyl group have a trans relationship. |
17.28 |
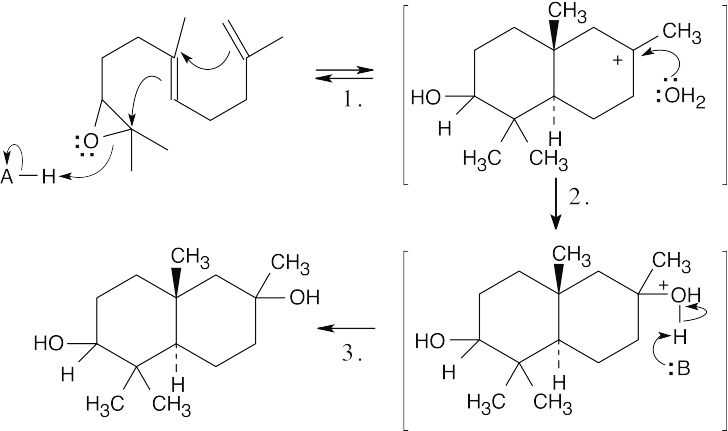
|
|
Step 1: |
Protonation. |
|
Step 2: |
Addition of H2O. |
|
Step 3: |
Loss of H+. |
17.29 |
Reaction of 2-butanone with NaBH4 produces a racemic mixture of (R)-2-butanol and (S)-2-butanol.
|
17.30 |
(a) |
Mechanism:
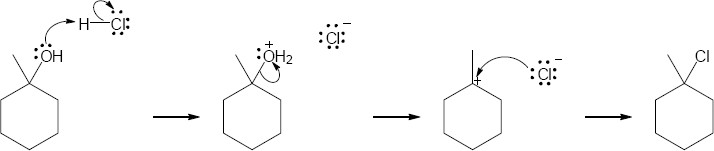
|
|
(b) |
Mechanism:

|
|
(c) |
Mechanism:

|
17.31 |
(a) |
Teritary alcohols undergo exclusively SN1 substitution.

|
|
(b) |
Reactions with PBr3 undergo SN2 substitution

|
|
(c) |
In this reaction the primary alcohol is first converted to a primary tosylate. Primary tosylates undergo exclusively SN2 substitution.

|
17.32 |
(a) |
Mechanism:

|
|
(b) |
Mechanism:

|
|
(c) |
Mechanism:

|
17.44 |
Grignard Reagent + Carbonyl Compound ⎯⎯⎯⎯⎯⎯⎯⎯→ Product (after dilute acid workup) |
|
(a) |
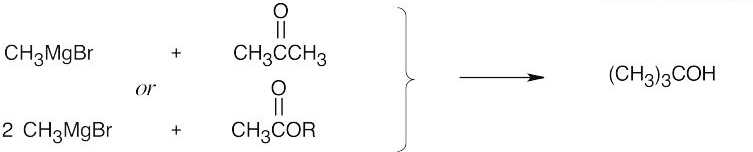 |
|
(b) |
 |
|
(c) |
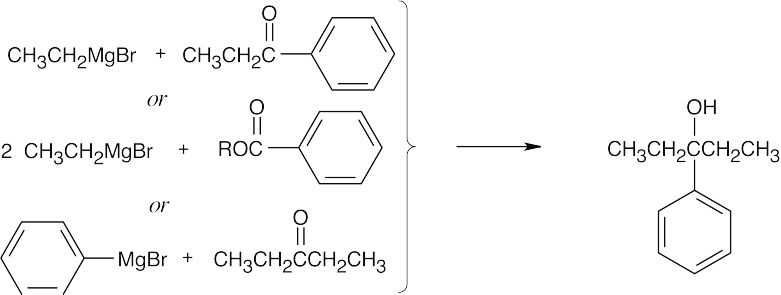 |
|
(d) |
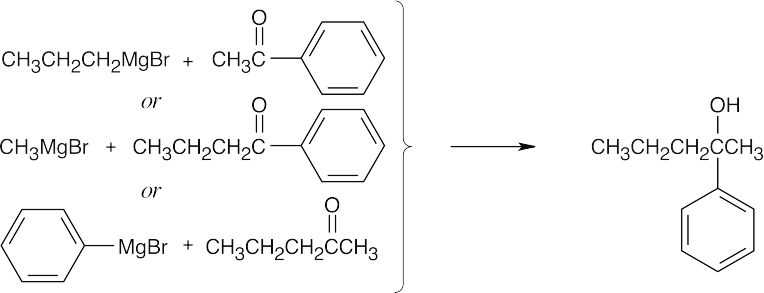 |
|
(e) |
 |
|
(f) |
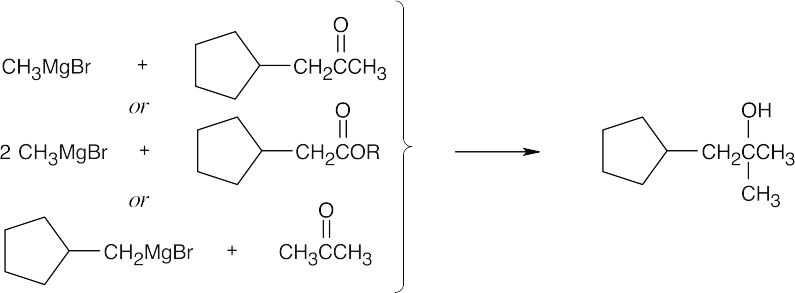 |
17.45 |
All of these syntheses involve a Grignard reaction at some step. Both the carbonyl compound and the Grignard reagent must be prepared from alcohols. |
|
(a) |
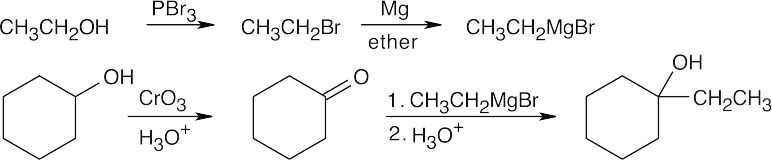 |
|
(b) |
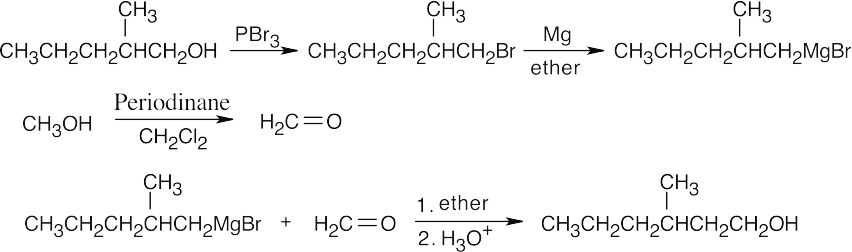 |
|
(c) |
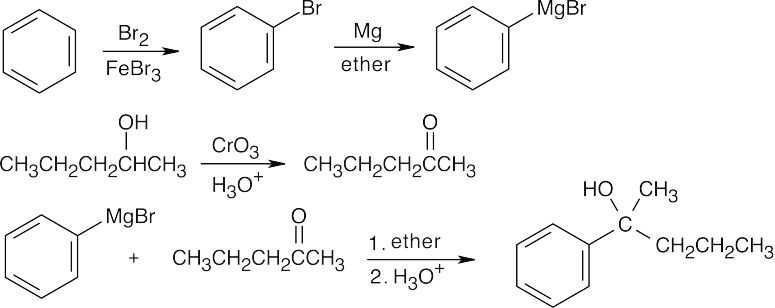 |
|
(d) |
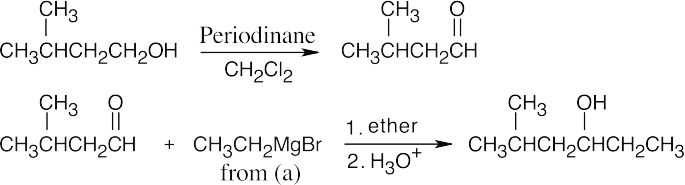 |
Reactions of Alcohols
17.47 |
(a) |
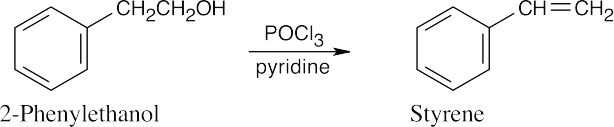 |
|
(b) |
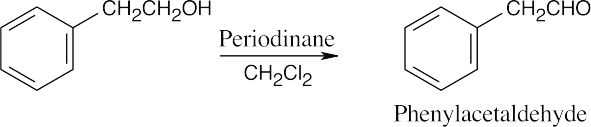 |
|
(c) |
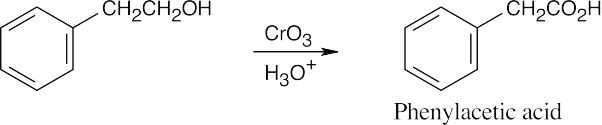 |
|
(d) |
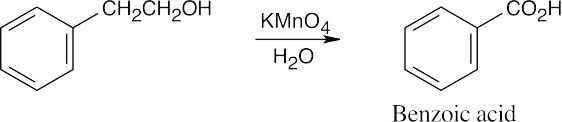 |
|
(e) |
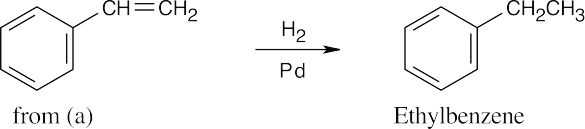 |
|
(f) |
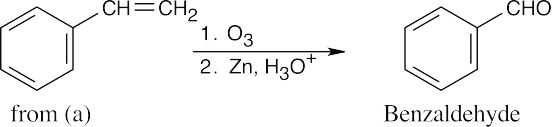 |
|
(g) |
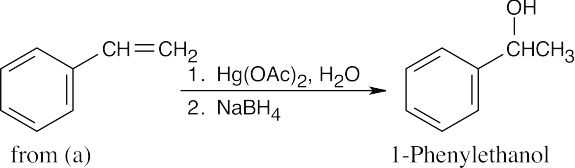 |
|
(h) |
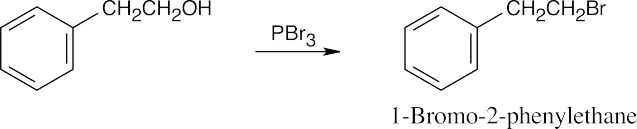 |
Spectroscopy
17.51 |
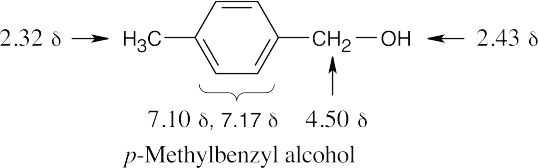
|
17.52 |
(a) |
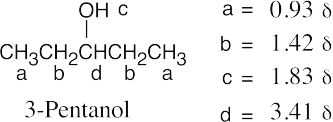 |
|
(b) |
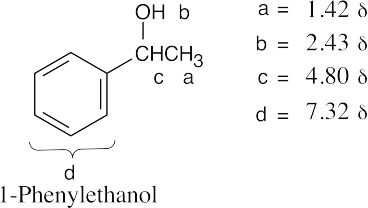 |
17.53 |
1. |
C8H18O2 has no double bonds or rings, based on degree of unsaturation. |
|
2. |
The IR band at 3350 cm–1 shows the presence of a hydroxyl group. |
|
3. |
The compound is symmetrical (simple NMR). |
|
4. |
There is no splitting.
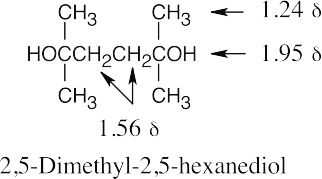
|
17.54 |
The peak absorbing at 1.76 δ (3 H) is due to the d protons. This peak, which occurs in the allylic region of the spectrum, is unsplit.
The peak absorbing at 2.13 δ (1 H) is due to the –OH proton a.
The peak absorbing at 2.30 δ (2 H) is due to protons c. The peak is a triplet because of splitting by the adjacent b protons.
The peak absorbing at 3.72 δ (2 H) is due to the b protons. The adjacent oxygen causes the peak to be downfield, and the adjacent –CH2– group splits the peak into a triplet.
The peaks at 4.79 δ and 4.85 δ (2 H) are due to protons e and f.
|
17.55 |
(a) |
C5H12O, C4H8O2, C3H4O3 |
|
(b) |
The 1H NMR data show that the compound has twelve protons. |
|
(c) |
The IR absorption at 3600 cm–1 shows that the compound is an alcohol. |
|
(d) |
The compound contains five carbons, two of which are identical. |
|
(e) |
C5H12O is the molecular formula of the compound. |
|
(f), (g) |
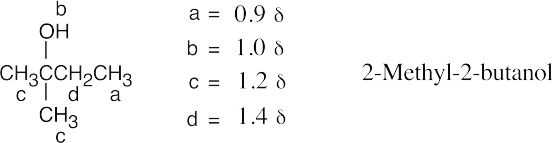 |
17.56 |
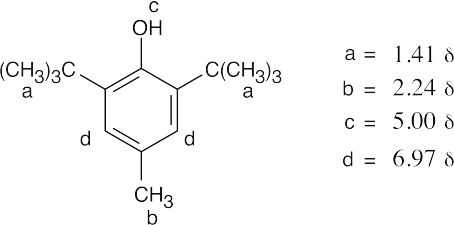 |
17.61 |
Despite this problem’s resemblance to Problem 17.24, the stereochemical outcome is different. Addition of methylmagnesium bromide to the carbonyl group doesn’t produce a new chirality center and doesn’t affect the chirality center already present. The product is pure (S)-2,3-dimethyl-2-pentanol, which is optically active.
|
17.62 |
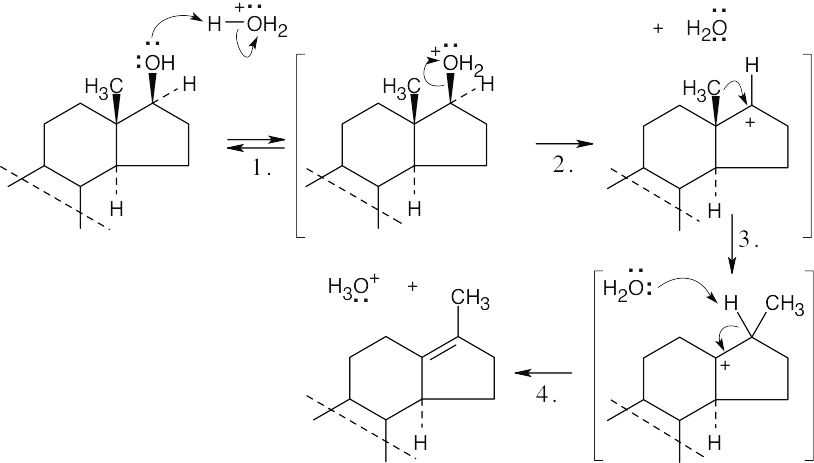
|
|
Step 1: |
Protonation. |
|
Step 2: |
Loss of H2O. |
|
Step 3: |
Alkyl shift. |
|
Step 4: |
Loss of H+. |
|
This is a carbocation rearrangement involving the shift of an alkyl group. The sequence of steps is the same as those seen in Problems 17.27 and 17.28.
|
17.63 |
All of these transformations require the proper sequence of oxidations and reductions. In (d), NaBH4 can also be used for reduction. |
17.64 |
A phenoxide anion is stabilized by the electron-withdrawing resonance effect of a p-nitro group. Methyl groups ortho to the phenol have no effect on acidity, but the methyl groups that flank the nitro group of the 3,5-isomer force the nitro group out of planarity with the ring and reduce orbital overlap with the π orbitals of the ring. The resonance stabilization of the nitro group is reduced, and the pKa of the phenol becomes higher, indicating lower acidity. |
17.65 |
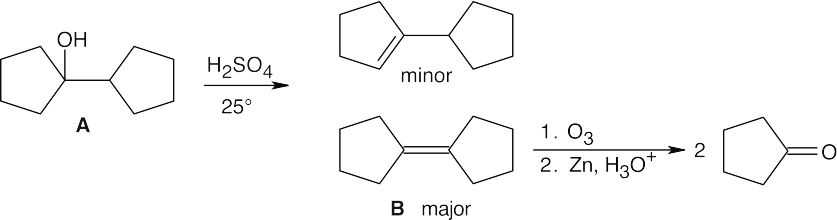 |
11.66 |
(a) |
Compound A has one double bond or ring. |
|
(b) |
The infrared absorption at 3400 cm–1 indicates the presence of an alcohol. The weak absorption at 1640 cm–1 is due to a C=C stretch. |
|
(c) |
(1) |
The absorptions at 1.63 δ and 1.70 δ are due to unsplit methyl protons. Because the absorptions are shifted slightly downfield, the protons are adjacent to an unsaturated center. |
|
|
(2) |
The broad singlet at 3.83 δ is due to an alcohol proton. |
|
|
(3) |
The doublet at 4.15 δ is due to two protons bonded to a carbon bearing an electronegative atom (oxygen, in this case). |
|
|
(4) |
The proton absorbing at 5.70 δ is a vinylic proton. |
|
(d) |
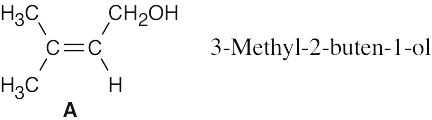 |
17.67 |
The more stable dehydration product is 1-methylcyclopentene, which can be formed only via syn elimination. The major product of anti elimination is 3-methylcyclopentene. Since this product predominates, the requirement of anti periplanar geometry must be more important than formation of the more stable product.
|
17.68 |
The pinacol rearrangement follows a sequence of steps similar to other rearrangements we have studied in this chapter. The second hydroxyl group assists in the alkyl shift.
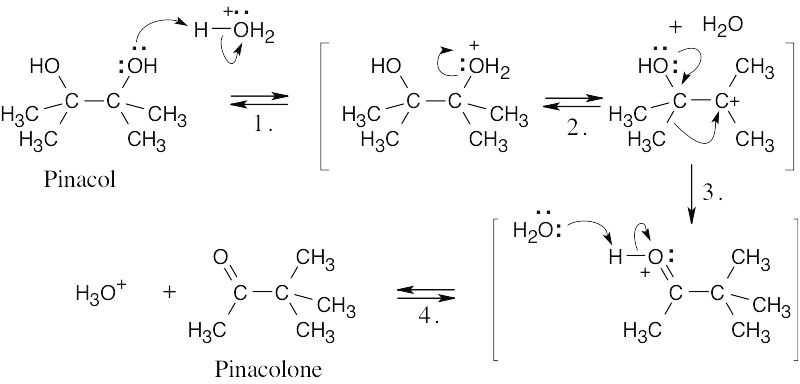
|
|
Step 1: |
Protonation. |
|
Step 2: |
Loss of H2O. |
|
Step 3: |
Alkyl shift. |
|
Step 4: |
Loss of H+. |
17.69 |
The hydroxyl group is axial in the cis isomer, which is expected to oxidize faster than the trans isomer. (Remember that the bulky tert-butyl group is always equatorial in the more stable isomer.)
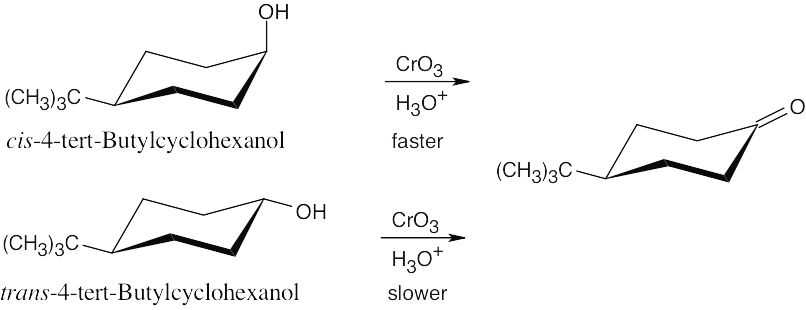
|
17.74 |
Structural formula: C8H10O contains 4 multiple bonds and/or rings.
Infrared: The broad band at 3500 cm–1 indicates a hydroxyl group. The absorptions at 1500 cm–1 and 1600 cm–1 are due to an aromatic ring. The absorption at 830 cm–1 shows that the ring is p-disubstituted. Compound A is probably a phenol.
1H NMR: The triplet at 1.16 δ (3 H) is coupled with the quartet at 2.55 δ (2 H). These two absorptions are due to an ethyl group.
The peaks at 6.74 δ-7.02 δ (4 H) are due to aromatic ring protons. The symmetrical splitting pattern of these peaks indicate that the aromatic ring is p-disubstituted.
The singlet absorption at 5.50 δ (1 H) is due to an –OH proton.
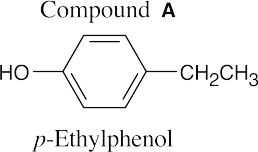
|
This file is copyright 2023, Rice University. All Rights Reserved.